It may be one of life’s greatest marvels—that underlying its diverse and myriad complexities are common building blocks shared by every extant lifeform. Of this evolutionary tendency to further develop the existing rather than creating anew, the eminent biologist and Nobel Laureate François Jacob famously wrote that “Nature is a tinkerer, not an inventor.” Still, it may come as a surprise to learn that underlying what is perhaps the pinnacle of evolutionary complexity—the human nervous system—are billions-of-years-old molecules that we share with organisms in every branch of the tree of life.
For more than 20 years, neurobiologist Tim Jegla has studied the evolution of these structures, known as ion channels, and their role in the nervous system. “These proteins,” he says, “are ancient. They go back, really, towards the beginnings of life.”
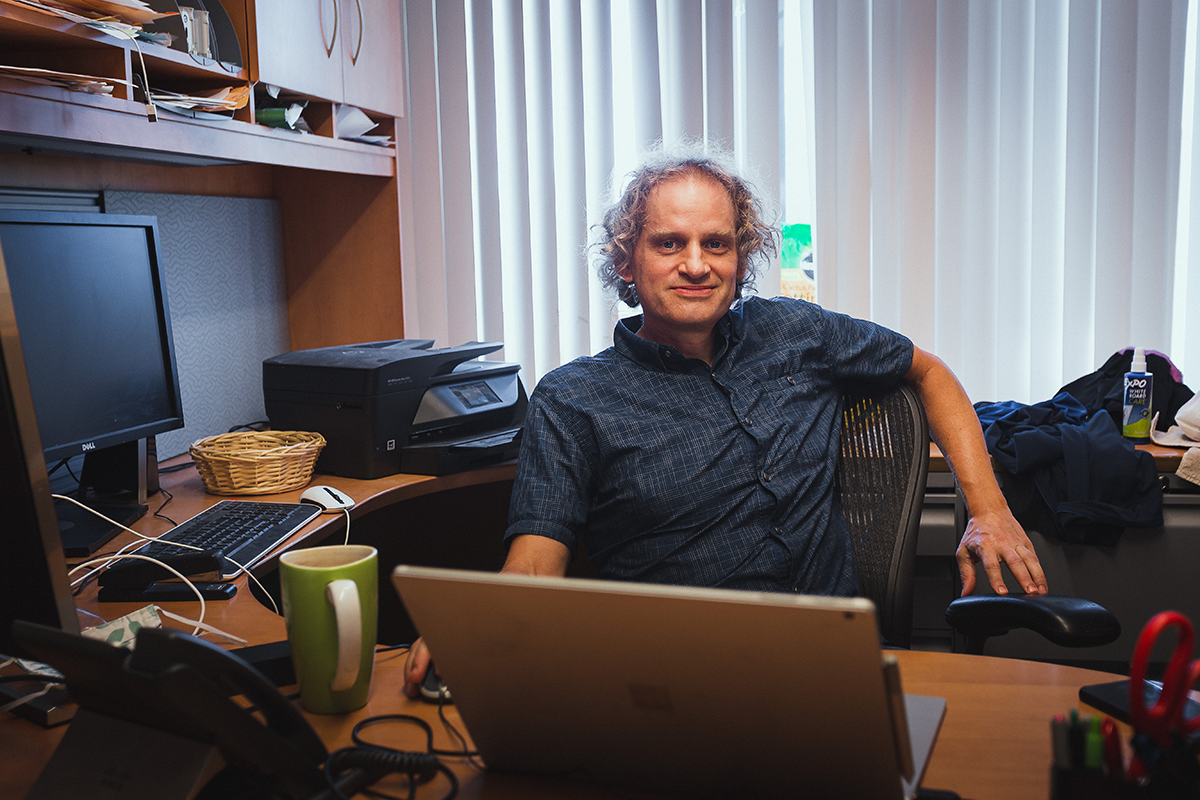
Ion channels are an integral component of the cell membrane, allowing charged particles (ions) to move in and out of the cell, thus creating electrical current—the basis of the signals that transmit information throughout the nervous system. In the hundreds of millions of years since the first ion channels were inherited by an ancestor common to all complex life on Earth and passed on to its diverging lineages, these proteins have continued to evolve along with us—“they’ve diversified to different ends,” Jegla says—but we still retain traces of their ancestral lineage in their present-day structures. “With new genome-based analyses of the evolutionary history of animals,” he explains, “we can see that a lot of that diversification in animals occurred after the evolution of the first nervous systems. But the diversification of this whole set of channels that we need to control electrical signaling in our complex nervous systems actually predates the evolution of complex nervous systems.”
With cell biologist Melissa Rolls, Jegla is trying to understand what evolutionary pressure drove this burst of molecular innovation roughly 600 million years ago.
What’s driven diversification
“The full diversity of neuronal channel types found in us isn’t there in the first nervous systems,” Jegla continues, “but it appears before we split from organisms like sea anemones that have very simple nervous systems. So why, in those very simple nervous systems, did you have all this molecular complexity? Essentially, why did you already have the molecular toolkit of complex, centralized nervous systems before those even appeared?”
Furthermore, very little innovation of that ancestral ion channel set has followed; they have remained relatively unchanged in the nearly 600 million years since, and Jegla hopes to find some insight into why that is. “We’ve evolved anatomical complexity and some specializations for space-saving, to pack more neurons in less space,” he says, “but the toolkit for modifying the behavior of the single neuronal cell seems to have been already there.”
Jegla hypothesizes that the diversification of ion channels was driven by the evolution of neuronal polarity—neurons with separate inputs and outputs (axons and dendrites), requiring different sets of channels for different positions in the cell—a crucial component of the nervous system’s centralization around the brain. “If that hypothesis is correct,” he says, “then we should find that the evolution of axons and dendrites occurred in animals with simple nervous systems, not later in complex bilaterians—animals with bilateral symmetry—like humans or even flies.” But to get to the bottom of this conundrum, Jegla needed the expertise of someone specializing in the evolution of neurons.
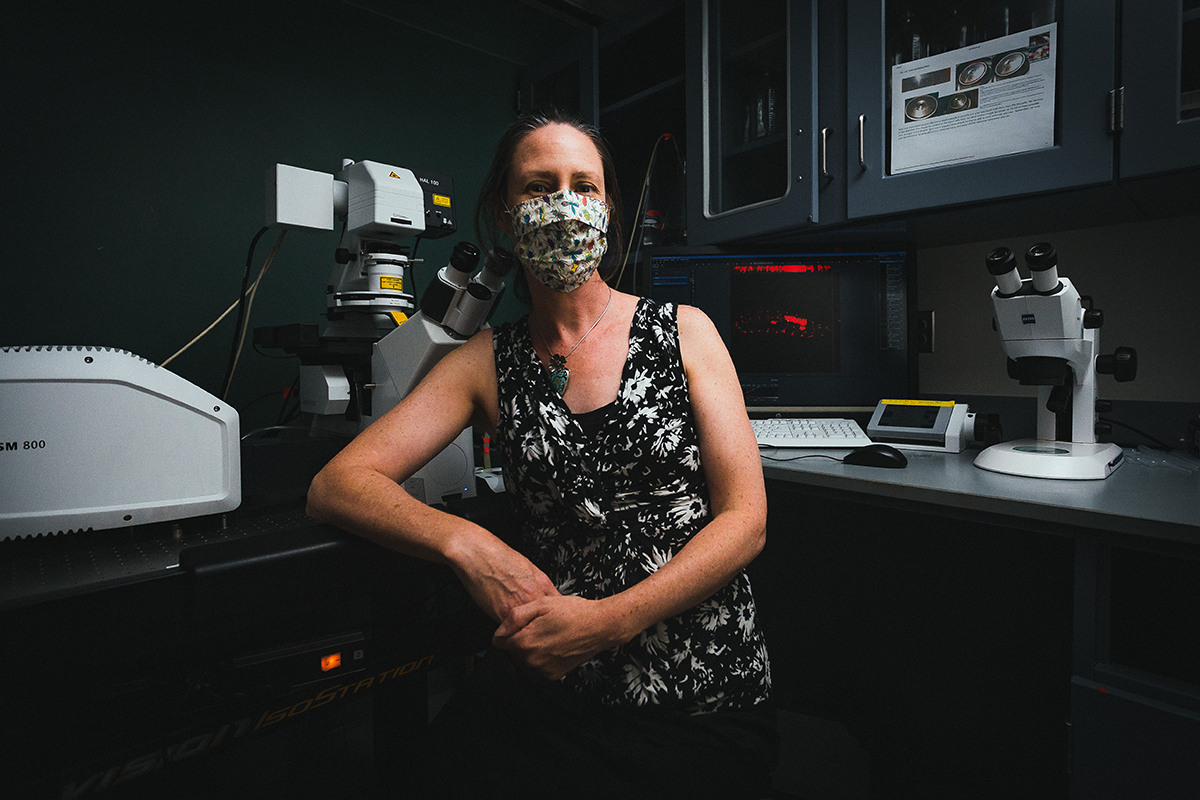
“We’ve been trying to understand whether neuronal polarity—having neurons with two different sides—evolved at the same time as centralization of the nervous system,” Rolls explains. “If you have a central nervous system, you have to have input, processing in the middle, and then output; so you need a lot of directional signaling to maintain that whole flow.”
Working with fruit flies and other model organisms, Rolls has characterized a number of differences between polar neurons’ axons and dendrites—the specialized structures that support directional signaling—and found that those basic features are shared by both vertebrates and invertebrates within the bilaterians. Based on that finding, she says, “It seems like the common ancestor of all bilaterians probably had neurons with well-defined dendrites and axons and the ability to send directional signals. But that common ancestor probably also had a central nervous system. And so the question is, ‘Did this differentiation of neurons with two compartments happen before that—and then was in place to allow centralization—or did it evolve only as the nervous system became centralized?’”
To answer that question, Rolls explains, requires examining the evolution of neurons outside the bilaterians, “and that means going to the next neighbor, which is cnidarians—things like sea anemones and hydra and jellyfish—which don't have a central nervous system; instead, they have a nerve net, and the cell biology of those neurons has not really been characterized.”
As Jegla identifies the building blocks—the ion channels, themselves—Rolls looks at how they’re assembled in the neuron. “What we've been doing together,” she says, “is to take that building block type—we look at the individual proteins and when they arose—and say, ‘Okay, how do those fit into the context of a cellular structure?’”
At the same time, with plant biologist Sally Assmann, Jegla is studying an ancient family of ion channels shared between animals and plants—looking for additional insight into the molecular mechanics of how ion channels function, which in turn will provide further insight into their evolution.
Further into form and function
Through a process known as gating, ion channels regulate the flow of ions across the cell membrane. All ion channels have some sort of gate, which opens and closes in response to different stimuli, but exactly how the physical gating is accomplished isn’t yet well understood.
With Assmann, Jegla is studying a unique family of highly diversified ion channels whose unusual gating plasticity may hold clues about an underlying mechanism. Although they are considered to be voltage-gated, these channels have evolved to respond to other stimuli, as well, such as molecular byproducts of cellular metabolism. In animals, they have diversified to underlie different types of sensory perception—including sight and smell—and to regulate heartbeat as well as help control electrical signaling in neurons. In plants, they have adapted to regulate osmotic gradients that open and close leaf pores during photosynthesis. “You can change how they're gated very easily,” Jegla says, “and so we want to know exactly how they're gated—on a molecular level, what makes them open and close—which allows them so easily to adapt to all these different physiological roles.”
Assmann explains that in plants, these ion channels are part of the membrane of specialized cells called guard cells, which surround the microscopic leaf pores (known as stomata) through which water and carbon dioxide are exchanged during photosynthesis. In response to various environmental signals, the guard cells can actually change their volume, thereby regulating the size of the stomata and the amount of water and carbon dioxide (CO2) that are exchanged. “When the plant, for example, is under drought stress,” she says, “the guard cells narrow those stomatal pores, and that limits water loss—albeit at the expense of CO2 uptake. But just like humans, plants can survive for a while without having food, but they can dry out pretty fast if they’re losing more water than they’re taking up from their roots.”
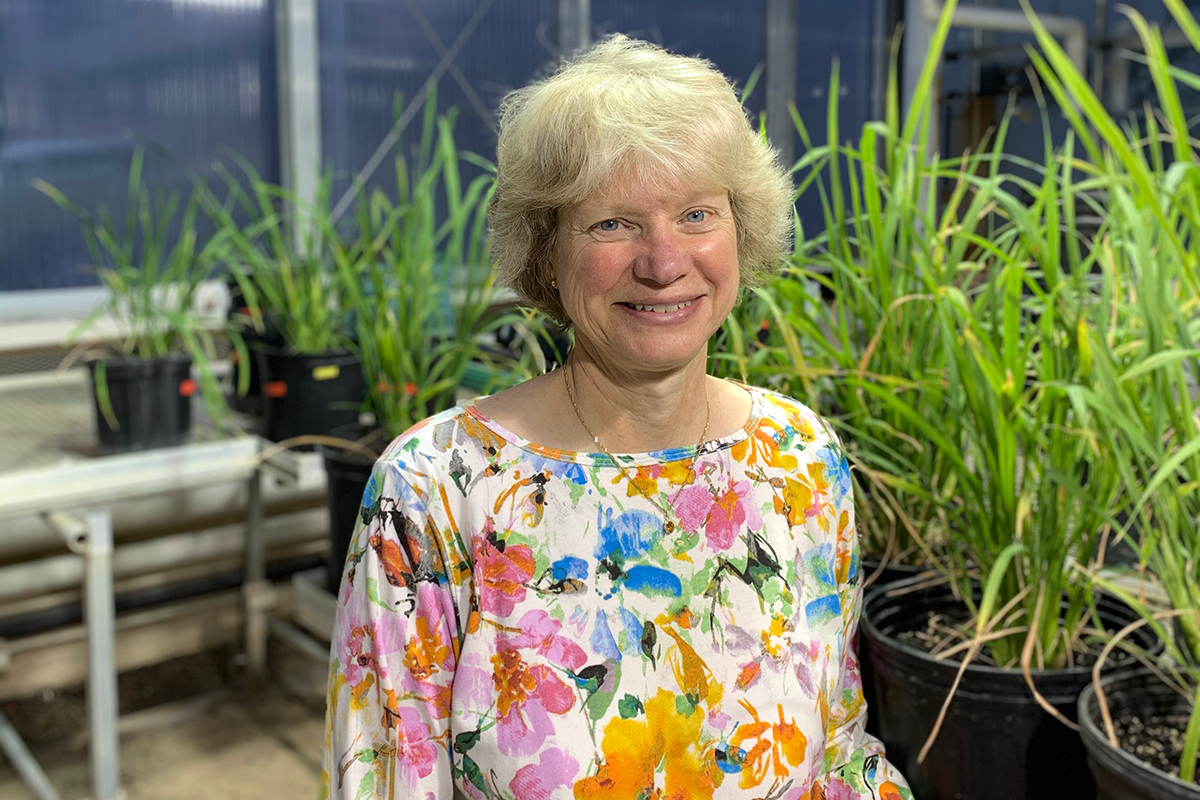
Examining the genetic sequences of the plant channels and comparing them with those of the animal channels—across the hundreds of amino acids that make up each channel protein—Jegla has found a conserved pattern of eight isolated amino acids spread throughout the channels. Out of those eight, he says, “we found one, in an unexpected place, that we hypothesize must be central to how the channels gate. But the larger motif—the conserved pattern—isn't understood at all. So we're trying to figure out how that all works.”
Looking farther down the road
As with all basic research, these collaborations aren’t being driven by a particular, practical problem or application so much as by the scientists’—and the scientific community’s—desire to contribute to understanding life more fully through these phenomena.
“As soon as you start to look at any process and really try to figure out what we actually know about it,” Rolls says, “you'll realize that we know only a small percentage. There will be a whole bunch of people working on understanding something in detail, and then there'll be this vast space where no one's ever even asked how does it work or what goes on there. So you end up with these little islands of knowledge in a sea of questions that haven't even been asked yet.”
But although these researchers aren’t seeking specific solutions, the knowledge arising from their studies will inform the work of other scientists who are.
For instance—better understanding neurons’ structure and function at a molecular level could lead to applications with neurodegenerative diseases, such as Alzheimer’s or Parkinson’s, or with neuronal injuries, such as those resulting from stroke or spinal cord damage. “Hopefully,” Rolls says, “we'll provide a framework for figuring out the mechanisms, structures, principles that allow neurons to survive and exist and function and do their job across these broad evolutionary time periods—and that can be useful in thinking about what goes wrong in disease or injury.”
Better knowledge of the mechanisms of plants’ drought-stress response could be a boon to plant breeders in the not-so-distant future. “With climate change and the global human population increase,” Assmann says, “there's already a demand for more stress-tolerant, more-resilient cultivars of our major food crops. So understanding this basic physiology is one of the first steps toward creating those improved varieties.”
And better knowledge of human ion channel function has a wealth of potential applications—particularly in the pharmaceutical industry, where it could inform the discovery of new drug targets for treating cardiovascular, metabolic, and neurological disorders—such as hypertension, type 2 diabetes, epilepsy, and stroke. “What you get out of understanding the gating mechanisms of these ion channels,” Jegla explains, “is strategies for modulating their activity—how you might be able to modify the channel with a drug to change its activity in very precise ways to get a therapeutic effect.”
There is also the possibility that a discovery in any one of these fields could cross over and lead to a groundbreaking result in another. “There are many similarities between all cells,” Assmann explains, “even though the macroscopic organism may look very different. It's possible that we can understand more about the functioning of a neuron by studying ion channels in a plant cell or vice versa.”
For today, though, it’s enough for Jegla to be searching for answers to fundamental questions.
“These broader discoveries, at times, lead us to very specific things,” he says. “But for me, what’s most motivating is that you’re learning important things about the history of life on Earth. I like that—it’s satisfying.”