A note from the guest editor:
It takes an inordinate curiosity to run a lab devoted to understanding basic biological processes and mechanisms, but it also takes an inordinate amount of work to keep abreast of the burgeoning literature that appears weekly in your area of research. It is even more difficult to be aware of the advances that are made in related fields of biology, and harder still to incorporate new data-acquisition and data-analytical techniques into the lab. How does a researcher do this? Well, it takes a village. It takes undergraduates, graduate students, a postdoc or two, and often a technician all of whom are motivated toward the same goal. It also takes a university with state-of-the-art core instrumentation. A faculty researcher in a laboratory science is often akin to an orchestral conductor who doesn’t play a single note but is the person most responsible for making beautiful music; or in this case of scientific research, advancing our understanding of the cellular processes of health and thereby advancing our understanding of the causes of a genetic disease. —Andy Stephenshon
The Science Underneath
White coats, test tubes, petri dishes, microscopes, and a burning curiosity to understand how things are connected and why they work. Long days and late nights in a starkly lit lab or in front of the blue-green glow of a computer monitor. Unglamorous as it is, this is where the quest to cure genetic disease begins.
“Modern medical advances get their start in labs; they are built on the foundation of fundamental scientific research,” said Douglas R. Cavener, Verne M. Willaman Dean of the Eberly College of Science at Penn State. “One of the main goals of our college is to advance science that improves human health, and we are doing this by pushing the boundaries of basic science with groundbreaking and innovative biomedical research. In my lab, we’ve been building this foundation over the past nearly two decades, working with a disease called Wolcott-Rallison syndrome, and we are now in a position to push to find a cure.”
Building a foundation
Curing diseases or developing new breakthrough treatments for previously intractable medical problems requires first understanding the biology that lies underneath. Disease-preventing vaccines rely on a basic understanding of the human immune system and pathogen biology. All modern genetic research is built on decades of fundamental research that led to the discovery of the doublehelix structure of DNA. Finding new and better treatments for the most pressing global health concerns of the 21st century—cancer, heart disease, diabetes, neurodegenerative disease, and infectious disease—will require an ever-more finely detailed understanding of the cellular, molecular, and genetic processes that govern human biology and how those processes can go wrong.
Much of this basic research is done in model organisms, such as fruit flies, roundworms, frogs, zebrafish, and mice. Although some, like the mouse, are more closely related to humans than others, none are human; but they provide the environments in which scientists can perform the experiments that allow them to start to piece together the fundamental processes that underlie human biology.
Another approach that has proved fruitful in advancing biomedical knowledge over the years is the use of immortalized human cell lines. Genetic changes and chemical treatments allow cells isolated from a variety of tissues and many cancers to be kept alive in the lab. Performing experiments on these human-derived cells in test tubes and petri dishes has its tradeoffs, of course. The cells are human, but they may behave differently when isolated in the lab as compared to how they would behave in a whole living organism. Model organisms, cell lines, and a host of experimental methods are the scientist’s toolkit with which they painstakingly build understanding of and lay the groundwork for eventual biomedical breakthroughs.
Genetic diseases are among the most complex in terms of finding a cure. When a person inherits a disease-causing genetic mutation from one or both parents, or if that mutation arises early in development, essentially every single cell in that person carries that mutation. By the time the disease can be diagnosed—occasionally prenatally, but usually at birth or even much later in life for genes that influence risk of cancer, heart disease, and others—fixing the problem may require finding a way to make changes in the genome of the millions of individual cells that make up the organs and tissues of the body.
A recently developed genetic technique is opening the possibility of doing just that. The CRISPR (pronounced “crisper”) -Cas9 system for genome editing allows researchers to make changes to genes in living organisms far faster and for far less money than ever before.
“As ways to deliver gene-correcting components have advanced, one can now contemplate correcting a genetic mutation in a large-enough number of cells in an organ to actually cure a disease,” said Cavener.
Building on the basic
Cavener is starting to take advantage of this new genome-editing technology, but getting to this point required years of basic research into Wolcott-Rallison syndrome. Wolcott-Rallison syndrome results in a suite of symptoms including infantonset diabetes, skeletal problems resulting from extremely low bone density, growth delay, and sometimes liver or kidney failure. It is caused recessive mutation in the PERK gene, and therefore defective copies of the gene must be inherited from both parents.
Though now the dean of the college, Cavener is a researcher at heart. He began his career in 1982 at Vanderbilt University, where he advanced from assistant to full professor. In 2000, he joined the Penn State Department of Biology as department head, where he maintained an active research program for 15 years. When he was appointed dean of the college in 2015, he had just gotten a major research grant renewed and had no intention of slowing down his research program.
“I’d learned to balance an active research program with my duties as department head, and as dean it’s beneficial for many reasons to stay involved in research,” said Cavener. “I’m busier, but I’ve got a great team of researchers in my lab, including Barb McGrath, a research associate professor, who has worked with me since I came to Penn State. In addition, my lab is in the Life Science Building right next door, so I can easily pop in to keep up with what’s going on.”
When Cavener started studying Wolcott-Rallison syndrome and the PERK gene in 2000, he had no idea that technology would be developed that might make it possible one day to cure the disease or at least one of the most debilitating aspects of the syndrome, infant-onset diabetes. He was interested in basic biology: How do cells regulate the production of proteins and deliver them to the appropriate places in the cell, and how does this process impact cell growth and division and the secretion of the hormones that control basic metabolic and neurological functions? Wolcott-Rallison syndrome provided a model to study these processes.
A fruitful approach to building an understanding of these basic biological functions is to study when something in the process goes wrong, like in a disease. If you want to understand a combustion engine without the benefit of an instruction manual, you could learn quite a bit by watching one that is purring along beautifully, but you might learn even more about the inner workings and interconnectedness of its various parts by diagnosing a worn-out spark plug or an oil leak. Likewise, through the course of history we have learned a lot about basic biology by studying genetic disease.
Rare diseases provide a disproportionate amount of information to their impact on society because they often result from mutations in a single gene that have major impact and can be tracked down by studying the families that carry them. Like diagnosing a relatively simple but severe problem like a broken piston in that engine instead of diagnosing a sputter that might be caused by any number of things. With only around 60 known cases, Wolcott-Rallison syndrome is a prime example.
The PERK gene that is responsible for Wolcott-Rallison syndrome codes for a protein kinase, an enzyme that modifies other proteins by attaching a chemical phosphate group to them. This addition alters the function of the newly modified protein, a process that is used in many cellular functions. Using a combination of traditional genetic, molecular, and cellular techniques, Cavener and his lab have worked out a number of details of the specific functions of the PERK kinase.
“Deciphering PERK’s function has not been an easy nut to crack,” said Cavener. “It actually has several different functions in many different places in the body, and discovering these multiple functions has been the major focus of my laboratory. We have a much better understanding of this complex protein than when we started, and its major function is much different than what people initially thought.”
Using traditional transgenic methods that allow researchers to manipulate the genome of a model organism, Cavener and his lab created a mouse model of Wolcott-Rallison syndrome in which the PERK gene is completely knocked out. They have also created conditional knock-out mice, where the PERK gene is removed from an individual tissue, and mice that express the PERK gene only in special tissues, allowing them to study its role in isolation from other dysfunctions caused when PERK is mutated in the whole body. These studies require an immense investment of time and resources, but by painstakingly studying the results of these experiments, Cavener and his group now understand many of PERK’s functions.
“What we found was that PERK is important in the regulation of intracellular calcium,” said Cavener. “Calcium ions are extremely important in cell signaling in pancreatic beta cells, the developing skeleton, and in the nervous system— another place where we see symptoms in Wolcott-Rallison syndrome.”
In a key experiment, Cavener and his lab group showed that they could completely reverse diabetes in their mouse model of Wolcott-Rallison syndrome. A mouse that expresses the PERK gene under the control of the genetic machinery that normally controls insulin expression in beta cells in the pancreas was bred with a mouse with no functional PERK gene of its own. The offspring, which then expressed PERK in the pancreatic beta cells but nowhere else, were completely cured of diabetes. Expressing PERK in these cells had rescued the ability of beta cells to produce and secrete insulin, which is severely limited in Wolcott-Rallison syndrome, allowing the mice to normally regulate blood-sugar levels.
With this basic biological knowledge built up over the years, Cavener has turned his attention to trying to cure the disease. Using the CRISPR-Cas9 gene-editing system, Cavener and his lab are developing the tools to fix the PERK gene in order to cure the infant-onset diabetes associated with Wolcott-Rallison syndrome, tools that he hopes can be applied more broadly to other genetic diseases.
Building a new gene
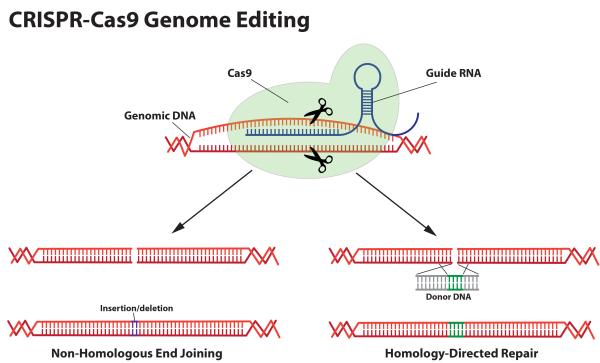
“I think we now know enough about PERK to think about how we can begin treating people who have this disease,” said Cavener, “and in doing so, develop treatments and methods that might be broadly applied to other human genetic diseases.”
Just as the rarity of Wolcott-Rallison syndrome made it a good candidate for identifying the PERK gene and deciphering its biology, aspects of how it leads to infant-onset diabetes in Wolcott-Rallison syndrome make it a good candidate for developing gene-editing techniques that could “cure” that part of the disease.
The infant-onset diabetes in Wolcott-Rallison syndrome results from a lack of insulin. This lack of insulin results from two defects in pancreatic beta cells: reduced cell proliferation during neonatal development and failure to process insulin through the secretory pathway. Inducing the expression of PERK specifically in beta cells in mice that otherwise lacked the PERK gene rescued this beta cell growth and insulin processing allowing the mice to produce enough insulin. Thus, if PERK expression could be restored to even some of the beta cells of patients with Wolcott-Rallison syndrome, it is likely that these beta cells would rapidly expand in number and secrete insulin to relieve the infant-onset diabetes.
“Just in the past four years, the CRISPR-Cas9 gene-editing technology has been developed and it has some enormous advantages over any previous methods for gene therapy,” said Cavener. “Previous to this, gene therapy did not seem like a realistic pathway for curing genetic diseases. But CRISPR gene editing came along and I quickly saw this as a game changer. You could really use this to correct mutations in humans after the disease was diagnosed, and restoring PERK function in pancreatic beta cells would be a good test case to develop the methods.”
CRISPR, which stands for “Clustered Regularly Interspaced Short Palindromic Repeats,” is a natural part of the defense system of bacteria against the viruses that attack them. Viruses that attack bacteria, known as bacteriophages, insert their DNA into the bacterial genome. The CRISPR system works by destroying the virus’s DNA as it enters the cell. The CRISPR locus in the bacterial genome stores small fragments of the virus’s DNA, which it uses to recognize the foreign DNA if the bacteria encounter it in the future. Surrounding these fragments of foreign DNA in the CRISPR locus are palindromic repeats (the DNA sequence of As, Ts, Cs, and Gs reads the same backward and forward, like “a man, a plan, a canal, Panama”). When these repeats and the virus-derived DNA they surround are transcribed, the resulting RNA folds on itself and forms a hairpin because the palindromic repeats can base-pair with each other. When the virus DNA at the head of the hairpin fi nds its match in a new invader, CRISPRassociated (abbreviated “Cas”) proteins, which are enzymes that cut DNA, are recruited to the site and the invading DNA is cut up and destroyed.
This system has now been co-opted and developed as the CRISPR-Cas9 system for genome editing. Hairpin-guide RNAs can be designed and built that target one of the bacterial Cas proteins, Cas9, to cut DNA very precisely at practically any location in the genome based on the DNA sequence. By adding the guide RNAs and Cas9 to human cells, researchers can direct the system to cut the DNA in the exact location that they want to edit. A variety of methods can then be used to edit the existing DNA, cut out unwanted segments, or insert new ones. The main limitations of the CRISPR-Cas9 system at the moment are effectively delivering it to cells and the efficiency with which the editing occurs. For some genetic diseases, such as Wolcott-Rallison syndrome, correcting the offending mutation in a small fraction of the cells should be sufficient to cure the disease; but in other cases, such as hereditary breast cancer, it may require correcting virtually all of the cells in breast tissue to avoid the cancer.
Jingjie Hu, a talented graduate student in the Cavener lab, has developed two parallel CRISPR gene-editing strategies that she has tested successfully in cell culture and shown to correct PERK mutations and restore function. The next and most challenging step will be to test these strategies in PERK-knockout mice. Once they show that they Feature Story can safely and effectively restore insulin production in the mouse model by repairing its PERK gene, they will look to gain approvals and implement their gene-editing technique to treat humans with Wolcott-Rallison syndrome.
“Although Wolcott-Rallison syndrome is extremely rare, having a treatment for the very worst of its symptoms would provide a great deal of relief and improve the lives of those who have it,” said Cavener. “But additionally, we hope that the methods that we develop will be more broadly applicable to other human genetic diseases and that, eventually, advances will allow us to start to address more common health concerns.”
Building for the future
Cavener sees the potential of the CRISPR-Cas9 system to revolutionize medicine. To further explore this potential, he and other scientists from the Huck Institutes of the Life Sciences and the Department of Biomedical Engineering at Penn State are in the process of developing a Center for Regenerative and Genetic Medicine. This new center would draw on faculty from across the University with the goal of advancing research in new gene-editing techniques like CRISPR-Cas9 in combination with stem cell research.
“I believe this will be the first center of its kind,” said Cavener. “Bringing together these two promising new technologies has the potential to cure some of the most pernicious human diseases.”
Part of the goals of the new center will be to ensure that Penn State has all of the necessary facilities, and recruits the best faculty and students, to be a leader in this burgeoning field. With these resources, Penn State will be able to continue to push the boundaries of biomedical science by continuing to do the hard work to understand the basic science that lies underneath and, most importantly, to translate these discoveries into cures for human diseases.